James Webb and Hubble Agree on Cosmic Expansion
The James Webb Space Telescope (JWST) has now confirmed earlier results from the Hubble Space Telescope (HST) regarding the universe’s expansion rate, refining the value of the Hubble Constant. This breakthrough contributes significantly to our understanding of cosmic distances and how the universe is expanding.
Summary
- The Hubble Constant (H0) measures the rate at which the universe is expanding.
- The constant is crucial for determining the age, size, and fate of the universe.
- Edwin Hubble first introduced the concept of an expanding universe in 1929.
- Recent research led by Adam G. Riess validates HST’s previous measurements using JWST.
- JWST’s analysis employs standard candles like Cepheid variable stars and Type Ia supernovae.
- The new value of H0 determined by JWST is 72.6 ± 2.0 km/s/Mpc, similar to HST’s 72.8 km/s/Mpc.
- The quest to resolve “Hubble Tension” continues, as various methods yield slightly different results.
- Further investigations include techniques using red giant branch stars and carbon-rich stars as distance indicators.
- Standard candles provide a robust way of measuring distances in the universe.
- Determining a precise value for H0 will help scientists better understand cosmic history.
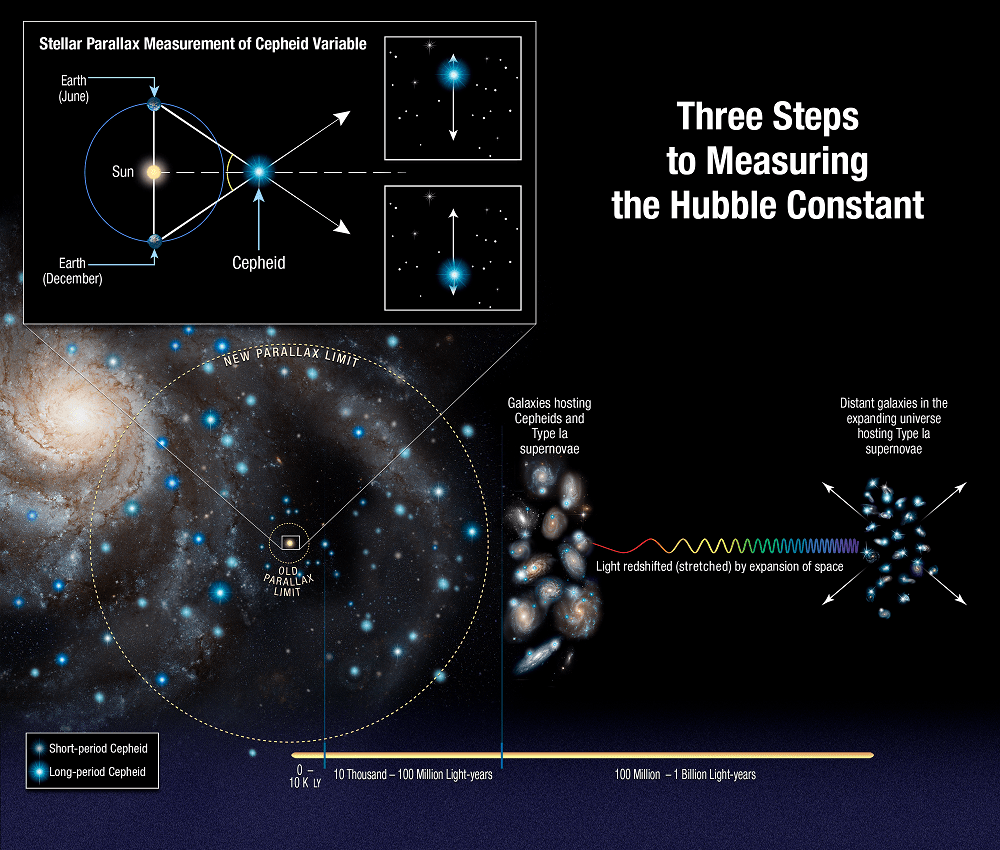
The latest Hubble study looked at more Cepheid variable stars. Cepheid variable stars are stars that change in brightness in a regular pattern. Astronomers used these stars to measure distances more accurately. They extended these measurements to distances up to 10 times farther across our galaxy than in the past. Credits go to NASA, ESA, A. Feild (STScI), and A. Riess (STScI/JHU).
Main Article
The universe is expanding, and at the core of this discovery is the Hubble Constant (H0), a critical cosmological value. The recent collaboration between the Hubble Space Telescope (HST) and the James Webb Space Telescope (JWST) has brought us closer to pinpointing the exact rate of cosmic expansion. This article explores the science, implications, and ongoing quest to resolve discrepancies in our understanding of the universe’s expansion rate.
The Hubble Constant (H0) describes the speed at which galaxies are receding from Earth, illustrating the universe’s continuous expansion. Edwin Hubble first calculated this in 1929, changing our understanding of cosmology forever. The value is expressed in units of kilometers per second per megaparsec (km/s/Mpc). A higher H0 means a younger universe, while a lower H0 implies an older one.
The challenge has always been achieving a high degree of precision. Small errors in measurement can lead to vastly different interpretations of the universe’s timeline.

The James Webb Space Telescope, managed by NASA, found a supernova in a faraway galaxy. This galaxy is named MRG-M0138. The telescope can capture multiple images of this supernova. Credit for the image goes to NASA, ESA, CSA, STScI, Justin Pierel from STScI, and Andrew Newman from the Carnegie Institution for Science.
The Role of Hubble Space Telescope
Since its launch in 1990, the Hubble Space Telescope has been instrumental in refining the Hubble Constant. By observing Cepheid variable stars—pulsating stars whose brightness fluctuates in a predictable pattern—HST has helped astronomers make significant advances. Cepheids serve as “standard candles,” objects with a known luminosity, allowing researchers to calculate distances accurately.
Moreover, HST has observed Type Ia supernovae, another class of standard candles. These supernovae occur in binary star systems and have a consistent peak brightness. By combining data from both Cepheids and supernovae, scientists have refined H0 over the years.
James Webb Space Telescope’s Contribution
The James Webb Space Telescope (JWST), launched in December 2021, provides a fresh perspective. Equipped with cutting-edge infrared technology, JWST can observe cosmic phenomena that HST cannot, such as stars shrouded in dust or galaxies in the distant universe.
The recent study led by Adam G. Riess from Johns Hopkins University uses JWST to validate HST’s previous findings. By examining Cepheids and Type Ia supernovae, JWST has derived a similar value for the Hubble Constant. The results are astonishingly close: 72.6 ± 2.0 km/s/Mpc, compared to HST’s 72.8 km/s/Mpc.
The Science of Standard Candles
Cepheid Variables
Cepheid variable stars are pulsating stars whose brightness variations occur in a regular, predictable manner. The period of pulsation is directly linked to the star’s intrinsic luminosity. By measuring the time it takes for the star’s brightness to vary, astronomers can determine its true luminosity and, subsequently, its distance from Earth.
Type Ia Supernovae
Type Ia supernovae are powerful explosions of white dwarf stars. They have a uniform peak brightness, making them ideal for measuring vast cosmic distances. When a white dwarf star accretes enough material from its companion, it reaches a critical mass, triggering a thermonuclear explosion. Observing these events has been key to understanding cosmic expansion.
Challenges and Hubble Tension
Despite advancements, determining H0 remains contentious. There is a persistent discrepancy known as Hubble Tension. This tension arises because different methods yield slightly different values for the Hubble Constant.
- Early Universe Measurements: Using the cosmic microwave background (CMB)—the afterglow of the Big Bang—H0 is estimated at around 67.4 km/s/Mpc. This is a lower value compared to results from standard candles.
- Late Universe Measurements: Observations of Cepheids and supernovae yield a higher H0, around 72–73 km/s/Mpc.
The inconsistency has led scientists to explore alternative theories, including potential modifications to the Lambda Cold Dark Matter (ΛCDM) model or the influence of new physics.
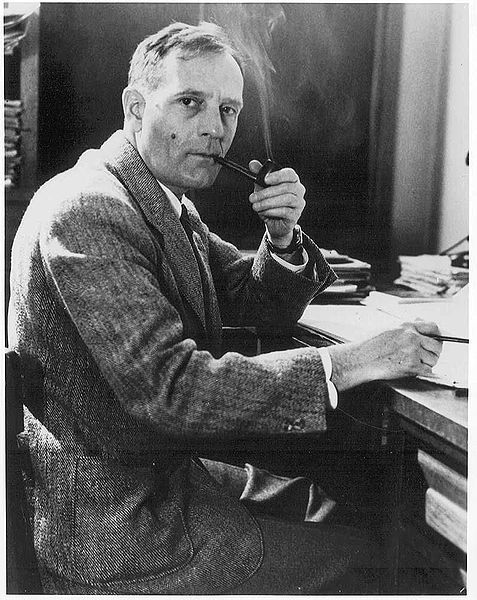
Methods to Measure Cosmic Expansion
Method | Description |
---|---|
Cepheid Variables | Pulsating stars with a predictable relationship between their brightness and pulsation period, used to measure distances to nearby galaxies. |
Type Ia Supernovae | Exploding white dwarfs with a uniform peak brightness, allowing accurate measurement of distances across vast cosmic scales. |
Cosmic Microwave Background (CMB) | The radiation left over from the Big Bang, used to calculate H0 based on observations of the universe’s early state. |
Technique | H0 Value (km/s/Mpc) |
---|---|
CMB Observations | ~67.4 |
Standard Candle Methods | ~72.6–73 |
Red Giant Branch Stars | Alternative standard candle method involving the luminosity of the brightest red giants in a galaxy. |
Implications of H0 for Cosmology
The exact value of H0 influences our understanding of several cosmic properties:
- Age of the Universe: The higher the value of H0, the younger the universe. Conversely, a lower H0 suggests an older universe.
- Size and Structure: The rate of expansion affects the large-scale structure of the universe, including galaxy clusters and cosmic voids.
- Dark Energy: The mysterious force driving the universe’s accelerated expansion remains a key area of study. A refined H0 can shed light on the nature of dark energy.
Ongoing Research and Future Prospects
The quest for an accurate Hubble Constant is far from over. JWST’s capabilities promise even more precise measurements. However, additional studies are needed to increase the sample size of supernovae and explore alternative methods, such as observing red giant branch stars and carbon-rich stars.
Astronomers also anticipate using the upcoming Roman Space Telescope to refine H0 further. The telescope will complement both HST and JWST, providing an independent verification of current measurements.
The agreement between Hubble and James Webb on the value of the Hubble Constant marks a significant milestone in cosmology. Yet, the Hubble Tension persists, and the quest to resolve it will drive scientific research for years to come. As technology advances, we may finally uncover the secrets of the universe’s expansion.
Facts About Cosmic Expansion
- Universe’s Age: Current H0 estimates suggest the universe is approximately 13.8 billion years old.
- Faster Than Light: Some galaxies appear to recede faster than light due to space expansion, not because they violate physics.
- Discovery of Cosmic Expansion: Edwin Hubble’s discovery built on Vesto Slipher’s earlier work on galaxy redshifts.
References
- Adam Riess’s Research on H0
- NASA’s Hubble Constant Findings
- James Webb Space Telescope Discoveries
- Planck Satellite Data on CMB