Essential Prebiotic Molecules Are Emerging in Space
Researchers have shown that key metabolic molecules, specifically the full set of carboxylic acids in the citric acid cycle, can form abiologically in the cold ices of interstellar clouds. This finding suggests that essential building blocks for life may have originated in space and been delivered to early planets, setting the stage for biochemical evolution on worlds like Earth.
Summary
- Laboratory simulations mimic interstellar molecular clouds at temperatures near 10 K and expose ice-coated dust analogs to ionizing radiation.
- Experiments produce all seven carboxylic acids of the citric acid (Krebs) cycle within a few million years.
- The study was conducted at the University of Hawaiʻi’s W. M. Keck Laboratory in Astrochemistry.
- Results published in Proceedings of the National Academy of Sciences demonstrate abiotic synthesis of metabolic intermediates.
- Simple molecules detected by JWST in interstellar ices (e.g., methane, methanol) serve as precursors.
- Cosmic rays drive chemistry on dust grains, forming mono-, di-, and tri-carboxylic acids.
- Laboratory abundances of some acids match those found in Ryugu asteroid samples returned by Hayabusa2.
- Findings support the idea that prebiotic chemistry predates planet formation.
- Abiotic citric acid cycle molecules could seed nascent solar systems via comets and asteroids.
- This work bridges astrophysics, chemistry, and biology in the context of life’s origins.
- Future missions and telescopes may detect more complex organics in space.
- Implications extend to the possibility of life’s chemistry emerging throughout the universe.
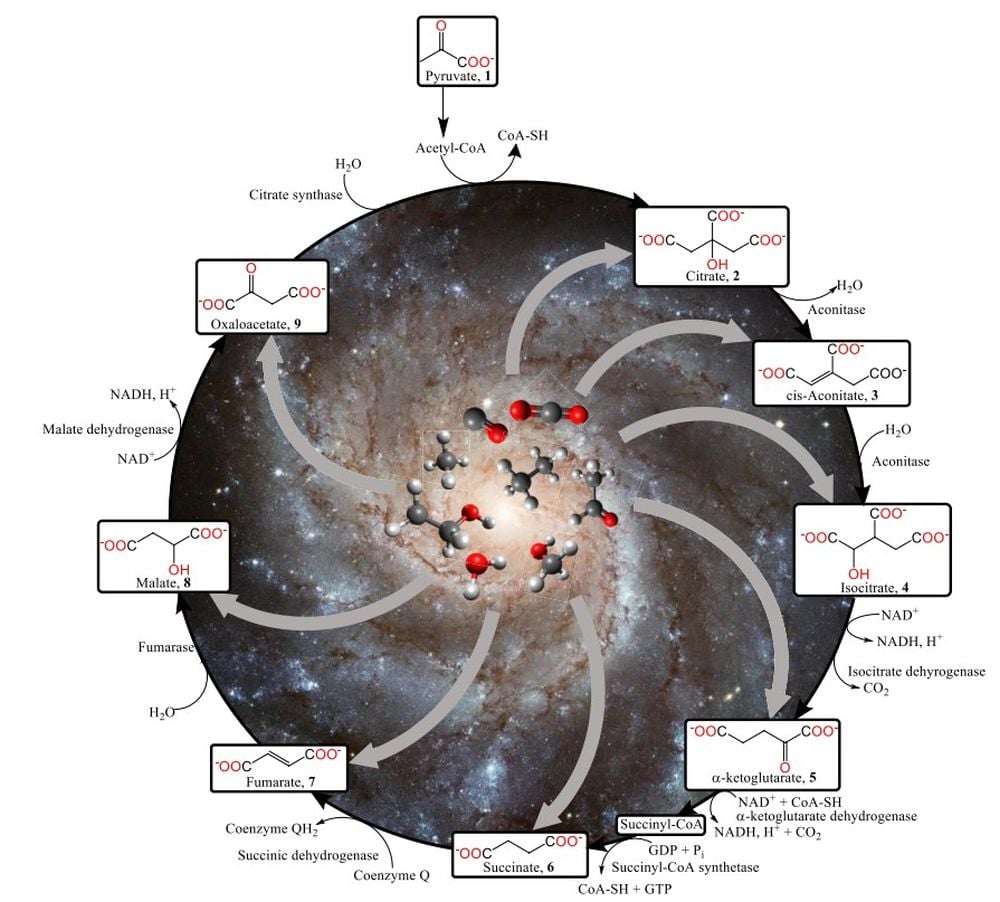
Introduction
The discovery that complex organic molecules can form in the harsh environment of deep space challenges the notion that life’s building blocks are unique to planets. Researchers have long studied how simple molecules like water and methane exist in interstellar clouds, but recent experiments reveal that even key metabolic intermediates can arise without biology. Understanding this process sheds light on how life’s chemistry may be universal rather than Earth-specific.
Background: Astrochemistry and Molecular Clouds
Astrochemistry explores how atoms and molecules interact in space, especially in dense molecular clouds that are the birthplaces of stars and planets. These clouds, with temperatures as low as 10 K, contain dust grains coated in icy mantles of simple molecules such as H₂O, CO₂, and CH₃OH. Cosmic radiation and ultraviolet light trigger reactions on these grains, producing increasingly complex organics over millions of years.
The citric acid cycle, also called the Krebs cycle, is central to energy production in aerobic life and involves a series of seven carboxylic acids that convert food into usable energy. Scientists have theorized that these molecules may have existed before life and were later incorporated into primitive metabolisms.
Laboratory Simulations of Interstellar Ices
In a recent study, researchers at the University of Hawaiʻi at Mānoa froze mixtures of simple gases to near absolute zero on nanoparticle dust analogs to simulate interstellar ices. They then bombarded these ices with high-energy particles mimicking galactic cosmic rays. After gradual warming, analyses revealed the formation of all seven carboxylic acids of the citric acid cycle within timeframes equivalent to a few million years in space.
Discovery of Citric Acid Cycle Precursors
Carboxylic Acid | Molecular Formula |
---|---|
Citric acid | C₆H₈O₇ |
Isocitric acid | C₆H₈O₇ |
α-Ketoglutaric acid | C₅H₆O₅ |
Succinic acid | C₄H₆O₄ |
Fumaric acid | C₄H₄O₄ |
Malic acid | C₄H₆O₅ |
Oxaloacetic acid | C₄H₄O₅ |
Table 1. Carboxylic acids of the citric acid cycle produced in interstellar ice simulations.
The experiments yielded mono-, di-, and tri-carboxylic acids matching the intermediates in the Krebs cycle. Some abundances closely align with measurements from Ryugu asteroid samples returned by JAXA’s Hayabusa2 mission, suggesting similar chemistry occurred in that body.
Implications for the Origin of Life
These findings imply that prebiotic metabolic molecules could form long before planets existed, riding on dust grains into emerging solar systems. If asteroids and comets delivered these organics to early Earth, they may have jump-started primitive metabolic networks that led to life.
“This work shows that the basic ingredients for life’s chemistry could have been made in space, long before Earth even formed,” said Professor Ralf I. Kaiser. “By simulating these deep space environments right here in Hawaiʻi, UH scientists are helping uncover how life might start not just on Earth, but anywhere in the universe.” Universe Today
Delivery to Early Earth
Once formed, these carboxylic acids can adhere to dust grains and become incorporated into comets and asteroids. When these bodies impact a young planet, they release their organic cargo, potentially seeding nascent worlds with metabolic precursors. Such delivery mechanisms are supported by analyses of meteorites, which reveal organic acids and amino acids formed extraterrestrially.
Future Research Directions
Further work will explore whether even more complex biomolecules, such as nucleobases or peptides, can form under similar space conditions. Next-generation telescopes like JWST may detect signatures of these compounds in interstellar ices, providing direct astronomical evidence. Understanding non-diffusive grain-surface chemistry could refine models of molecule formation in cold cores and protoplanetary disks.
Facts
- Space dust grains can be smaller than a human cell yet host complex chemistry.
- The citric acid cycle was first described by Hans Krebs in 1937, earning him a Nobel Prize in 1953.
- Some cometary ices contain amino acids, the building blocks of proteins.
- Galactic cosmic rays travel at nearly the speed of light and drive space chemistry.
- Over 200 different molecules have been detected in the interstellar medium.
Source | Abundance Ratio |
---|---|
Laboratory ice simulation | 1.0 (normalized) |
Ryugu asteroid sample | 0.8–1.2 (for key acids) |
Table 2. Comparison of relative abundances for select carboxylic acids between laboratory simulations and Ryugu samples.
References
- Mason McAnally et al., “Abiotic origin of the citric acid cycle intermediates,” PNAS, 2025. PNAS
- “Prebiotic Molecules are Forming in Space,” Universe Today, April 24, 2025. Universe Today
- PubMed, “Abiotic origin of the citric acid cycle intermediates,” PMID: 40258155. PubMed
- University of Hawaiʻi news release, “Scientists recreate deep space chemistry linked to first metabolic systems on Earth,” April 21, 2025. University of Hawaii
- “Scientists recreate deep space chemistry linked to first metabolic …,” Phys.org, April 2025. Phys.org
- “Molecules From Space May Have Sparked Life on Earth Billions of Years Ago,” Discover Magazine. Discover Magazine
- Wikipedia, “Astrochemistry.” Wikipedia
- Wikipedia, “Molecular cloud.” Wikipedia
- Simple English Wikipedia, “Krebs cycle.” Simple English Wikipedia
- Wikipedia, “Citric acid cycle.” Wikipedia
- University of Hawaiʻi news, “Cool Sugar Acid Formation in Space,” March 2024. University of Hawaii
- ArXiv, “Formation of Complex Organic Molecules in Cold Interstellar Environments,” Jin & Garrod, 2020. arXiv
- Wikipedia, “List of interstellar and circumstellar molecules.” Wikipedia
- Wikipedia, “Citric acid.” Wikipedia
- Wikipedia, “Interstellar ice.” Wikipedia